Application Note
TheWell Bioscience Inc.
Introduction
Mesenchymal stem cells are a type of adult multipotent stem cell that gives rise to bone, cartilage, adipose, and connective tissue. Over the last two decades, they have become a wildly popular potential alternative to embryonic stem cells for regenerative medicine because of their broad plasticity, immunomodulatory, and anti-inflammatory properties, and the relative ease in which they can be isolated1. In vitro, they have been repeatedly demonstrated to be capable of generating various non-mesodermal and non-mesenchymal tissues including hepatocytes2,3, cardiomyocytes4, pancreatic cells5, neuronal cells6, and melanocytes7. They can be isolated with reasonable abundance and in a patient-specific fashion from adipose tissue, bone marrow, cord blood, and synovial fluid8-10. Their immunomodulatory abilities make them popular not only because they hold promise for possible treatments for previously difficult-to-treat diseases such as graft-versus-host disease and for organ transplantation, but also because any tissues derived from MSCs will themselves engender little to no immune response compared to iPSC- and ESC-derived tissues11.
However, for all of their therapeutic potential, they have one major setback: in vitro, their proliferation rate tends to slow drastically, and they begin to senesce readily, making them difficult to expand enough for therapeutic use12. Some studies have suggested that adjusting the glucose concentration in the culture media can mitigate some of the senescence13, but this means culturing the cells in an environment that is not clinically relevant and which could ultimately alter their genetic stability. Studies have been conducted on the effect of 2D cell culture on the expression patterns and they have found that 2D culture tends to result in the overall flattening of the cytoskeleton and nucleus which in turn results in a change in gene and gene expression and rearrangement of the extracellular matrix. Three-dimensional cultures have proven to be much more successful in maintain the ECM microenvironment and produce more uniform, reproducible results and have demonstrated more success with clinical applications including transplantation.
OP9 cells are a characterized line of murine bone marrow stromal cells that do not produce functional macrophage colony-stimulating factor (M-CSF) due to an osteopetrotic mutation in the gene encoding M-CSF14. OP9 cells were initially used to coculture mouse embryonic stem cells to induce their differentiation into blood cells, but they have been characterized as mesenchymal stem cells15. Here we discuss culturing of 3D Cell Culture of OP9 on VitroGel® hydrogel system including the VitroGel 3D-RGD and the new VitroGel RGD-PLUS, which contain different levels of integrin binding ligand modifications (RGD peptide) for different cell-cell and cell-matrix interactions. (VitroGel 3D-RGD is a ready-to-use tunable hydrogel system modified with RGD cell adhesive peptide, promoting the cell attachment and cell-matrix interactions during the 3D cell culture. VitroGel RGD-PLUS is modified with 3X higher concentration of RGD peptide compared to regular VitroGel 3D-RGD, which maintain a high level of integrin binding activities even after hydrogel dilution, supporting cells requiring strong cell-cell and cell-matrix interactions).
Materials and Methods
Cell Culture
OP9 cells were maintained in Alpha Minimum Essential Medium supplemented with 10% FBS and 1x pen-strep. Cells were passaged when the cultures reached 80-90% confluence. For 2D hydrogel coating culture and 3D cell culture, both VitroGel 3D-RGD and VitroGel RGD-PLUS were used, according to the user handbook, as described below.
2D coating culture method
2D coating hydrogel was prepared at 1:0, 1:1 and 1:3 dilutions with VitroGel Dilution Solution (Type 1) and then mixed with OP9 medium without cells at 4:1 ratio; 300μl added to each 24-well plate. The hydrogel was stabilized at room temperature for 20 minutes, then cells were added on the top of the hydrogel suspended in 300μl of cover medium. Medium was changed every other day.
3D coating culture method
Hydrogel was prepared at a 1:3 dilution with VitroGel Dilution Solution (Type 1) according to manufacturer protocol. For confocal/fluorescent imaging, cells were cultured 8 well chambers with cover glass on the bottom, using 200μl hydrogel per well. Medium was changed every other day.
Immunofluorescent (IF) Imaging
Since cells have a GFP reporter, we conducted live cell imaging. The images were taken using a Leica TCS SP8 scanning confocal microscope with z-stack function at 10X and 20X magnification and processed by LAS X software and ImageJ.microscope with z-stack function. Processing and analysis of images were performed using LAS X software and ImageJ.
Results
OP9 cells in 2D VitroGel 2D culture exhibit cell clustering and healthy cell morphology.
We evaluated OP9 cells in 2D culture in both the original VitroGel 3D-RGD and the new VitroGel RGD-PLUS. In standard 2D culture on the surface of tissue culture plate, OP9 cells like many mesenchymal cell types, adopt a fibroblast like shape and fail to cluster (Fig 1A). Mesenchymal stem cells in this environment don’t proliferate well and can lead to senescence. Moreover, they exhibit the characteristic bright spotting in the cell culture commonly associated with stages of apoptosis. To understand optimal conditions for OP9 culture within our tunable system, we cultured these cells various dilutions of VitroGel. In the VitroGel 3D-RGD (Fig 1B-D), cells form clusters in all dilutions after just 2 days of cell culture. With the newer VitroGel RGD-PLUS (Fig 1E-G), cells are even more robust, forming large colonies and exhibiting a healthy MSC morphology at the lowest dilution.
Figure 1: OP9-GFP cells grown on the top of 2D hydrogel coating surface of VitroGel 3D-RGD and VitroGel RGD-PLUS. Compared to standard 2D culture (A), OP9 cells attach and cluster much better in the 3D VitroGel environment (B-G) after only 2 days in culture Cells show aggregation at 1:0 dilution for both VitroGel products (B, E), but the cells spread and attach better on VitroGel RGD-PLUS at lower gel strength substance.
Figure 2: Three-dimensional culture of OP9-GFP cells in both VitroGel 3D-RGD and VitroGel RGD-PLUS. While VitroGel 3D-RGD promotes cell expansion (E,G), the new VitroGel RGD-PLUS has better support for OP9 cell proliferation and cell-cell communication. Stronger cell-matrix interactions help the cells to form the cell-networking structures (F,H). (images A,B,C,D,G,H at 20X; images E&F at 10X).
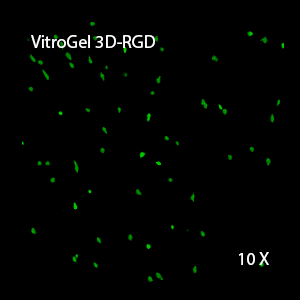
3D View of E
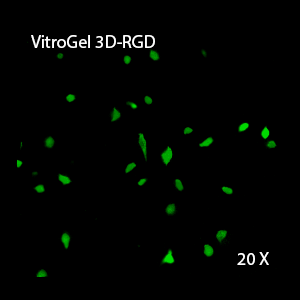
3D View of G
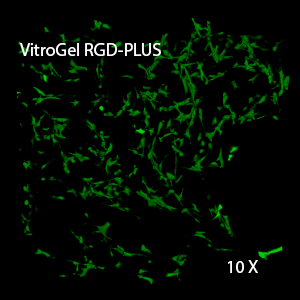
3D View of F
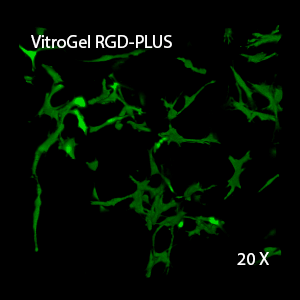
3D View of H
Culturing OP9 cells in VitroGel RGD-PLUS promotes the construction of an intercellular network
This characteristic is typically not possible in two-dimensional culture and can vary with three-dimensional culture. To understand whether our hydrogel system can facilitate this, we cultured OP9 cells in three-dimensional cultures with two of our hydrogels. Based on our previous experience, for 3D cell culture, OP9 cells prefer to grow in a soft hydrogel matrix (G’ < 300 Pa). Therefore, the diluted VitroGel hydrogels at 1:3 dilution has been selected for this study. A major factor of the in vivo 3D microenvironment is the ability of cells to form an intercellular network. With VitroGel 3D-RGD, after 7 days of culture, cells have settled into the 3D hydrogel (Fig 2C, E, G). The cells are alive, but the proliferation rate is low comparing based on Day 2 and Day 7 images (Fig 2A & C). The 3D views of the Fig 2E & G show the lack of intercellular connections of OP9 cells after 7 days 3D culture in regular VitroGel 3D-RGD. On the other hand, cells cultured in VitroGel RGD-PLUS have shown more 3D fibroblast like morphology and made extensive intercellular connections (Fig 2D, F, H; 3D Views of Fig 2F & H).
Discussion
Our studies indicate that our new VitroGel RGD-PLUS can be an ideal three-dimensional scaffold for cellular applications that rely on intercellular networks. Cellular differentiation and identity is regulated by complex cell-cell communication which can only be achieved at a short range by diffusible ligands; a behavior which can be difficult to model in vitro. It can lead to variability between in vitro and in vivo studies which can hinder translational studies16.
For tissue regeneration, especially mesenchymal differentiation, the ability to communicate with surrounding cells is absolutely necessary. Cell communication commonly happens at relatively short-range via diffusible factors between cells, facilitated by the close contact provided by a three-dimensional environment. However, longer range signals require a cell communications network. Multiple studies have demonstrated that different kinds of scaffolds and different elasticities can drive differentiation down different pathways. VitroGel system provides a platform that is adjustable enough to fit any of the applications, reducing variability and streamlining the process.
Reference
- Ullah, I., Subbarao, R. B. & Rho, G. J. Human mesenchymal stem cells – current trends and future prospective. Biosci. Rep. 35, 1–18 (2015).
- Stock, P. et al. The generation of hepatocytes from mesenchymal stem cells and engraftment into murine liver. Nat Protoc 5, 617–627 (2010).
- Itaba, N. et al. Hepatic cell sheets engineered from human mesenchymal stem cells with a single small molecule compound IC-2 ameliorate acute liver injury in mice. Regen Ther 9, 45–57 (2018).
- Arslan, Y. E., Galata, Y. F., Sezgin Arslan, T. & Derkus, B. Trans-differentiation of human adipose-derived mesenchymal stem cells into cardiomyocyte-like cells on decellularized bovine myocardial extracellular matrix-based films. J Mater Sci Mater Med 29, 127 (2018).
- Belame Shivakumar, S. et al. Pancreatic endocrine-like cells differentiated from human umbilical cords Wharton’s jelly mesenchymal stem cells using small molecules. J. Cell. Physiol. 234, 3933–3947 (2019).
- Srivastava, A. et al. Secretome of Differentiated PC12 Cells Enhances Neuronal Differentiation in Human Mesenchymal Stem Cells Via NGF-Like Mechanism. Mol. Neurobiol. 55, 8293–8305 (2018).
- Paino, F. et al. Ecto-mesenchymal stem cells from dental pulp are committed to differentiate into active melanocytes. Eur Cell Mater 20, 295–305 (2010).
- Jia, Z. et al. Isolation and characterization of human mesenchymal stem cells derived from synovial fluid by magnetic-activated cell sorting (MACS). Cell Biol. Int. 42, 262–271 (2018).
- Amati, E. et al. Generation of mesenchymal stromal cells from cord blood: evaluation of in vitro quality parameters prior to clinical use. Stem Cell Research & Therapy 2017 8:1 8, 14 (2017).
- Baer, P. C. Adipose-derived mesenchymal stromal/stem cells: An update on their phenotype in vivo and in vitro. World J Stem Cells 6, 256–265 (2014).
- Gao, F. et al. Mesenchymal stem cells and immunomodulation: current status and future prospects. Cell Death & Disease 7, e2062 (2016).
- McKee, C. & Chaudhry, G. R. Advances and challenges in stem cell culture. Colloids Surf B Biointerfaces 159, 62–77 (2017).
- Li, Y.-M. et al. Effects of high glucose on mesenchymal stem cell proliferation and differentiation. Biochem. Biophys. Res. Commun. 363, 209–215 (2007).
- Nakano, T., Kodama, H. & Honjo, T. Generation of lymphohematopoietic cells from embryonic stem cells in culture. Science 265, 1098–1101 (1994).
- Gao, J. et al. Characterization of OP9 as authentic mesenchymal stem cell line. J Genet Genomics 37, 475–482 (2010).
- Thurley, K., Wu, L. F. & Altschuler, S. J. Modeling Cell-to-Cell Communication Networks Using Response-Time Distributions. Cell Syst 6, 355–367.e5 (2018).